Key methodologies and our expertise
We offer EPR measurements at both low and room temperatures.
The low temperature EPR measurements is our main method of interrogating biological samples. For that, we currently use an Oxford Instrument helium system (Figure 2). Paramagnetic species are often transiently formed in biochemical reactions, often formed and gone over short time periods. To detect those, we freeze the reaction mixtures at specific times after the reaction is initiated. If such reactions are fast, the freezing time of the reaction mixture must be short.
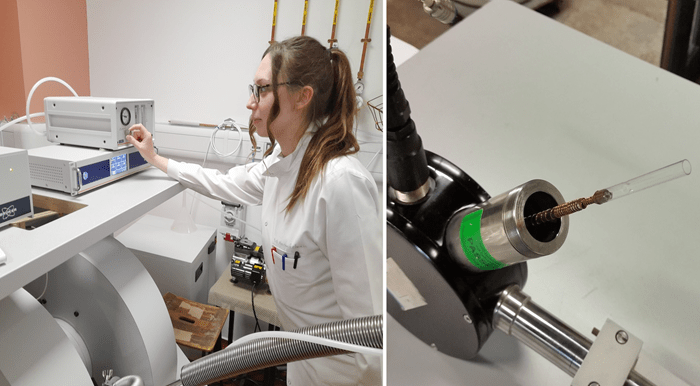
Figure 2: Left - Marina Rozman, PhD, is controlling the stability of the temperature set by the Oxford liquid helium system. Right – the heart of the cryostat – this is where the cold helium hits and cools the sample tube.
For the very short reaction times, we have a unique Rapid Freeze Quench (RFQ) apparatus (Figure 3) that allows freezing the reaction mixtures on a liquid nitrogen cooled metal surface. This makes our bespoke apparatus different from the most commonly used RFQ machines that employ isopentane as the cryogen (thus entailing a number of methodological challenges and outcome uncertainties). The University of Essex EIRA (Enabling Innovation: Research To Application) has produced a video of how our RFQ apparatuses might be used.
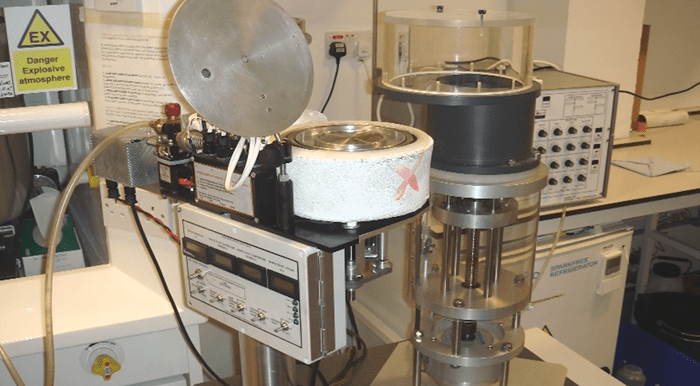
Figure 3: Our liquid nitrogen system to freeze quench samples together with the Update Instruments ram system to make solution mixtures.
Our RFQ apparatus can be operated in gas controlled conditions, for example – in an anaerobic environment (Figure 4).
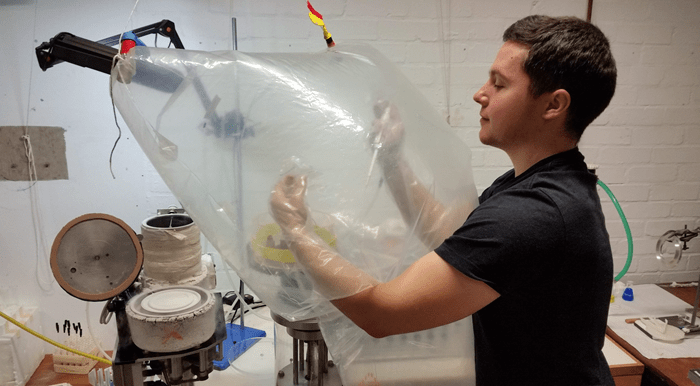
Figure 4: Jacob Pullin, PhD, operates the RFQ system in oxygen-free argon atmosphere.
We also use our EPR spectrometer at room temperature, when the samples are in the liquid phase. The useful gadget for that is the Bruker AquaX system. With our bespoke attachment to an AquaX (Figure 5, right top), we can deliver a mixture of two reaction components to the spectrometer resonator and monitor the EPR spectrum change over time, from as early as ~1 s after mixing. Figure 5, left, shows the room temperature EPR spectra of the mixtures of human haemoglobin and hydrogen peroxide as observed in four similar experiments, marked by different colours of the spectra [1]. A tyrosine free radical is formed and quickly disappears in the reaction – the four sets of spectra in the figure show only the initial time points when the free radical EPR spectra intensities are relatively high. But those intensities can be accurately quantified over the whole time course of the reaction – and that allows measurements of the Tyr free radical kinetics, as shown in Figure 5, right bottom.
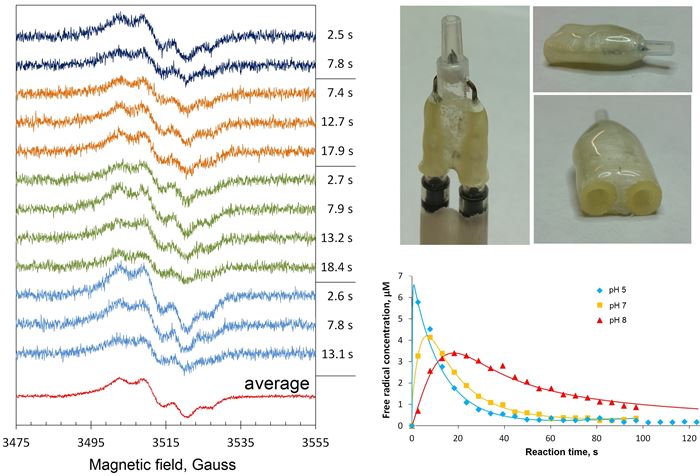
Figure 5: A bespoke attachment to the Bruker AquaX system, for measuring liquid samples, allows quantification of spectral change with a few seconds resolution. This allows accurate kinetic dependences measurements.
The liquid phase measurements at room temperature is particularly useful for the studies of protein spin labels and spin traps of protein-bound radicals and paramagnetic reactive oxygen species.